Most people have never heard of vacuum decay, but if it happened it would be the biggest natural disaster in the universe. Sure, an asteroid could destroy a city or wipe out life on Earth. A supernova could fry the ozone layer. If a blast of energy from a spinning black hole hit our planet, it could rip apart the entire solar system. As dramatic as these disasters are, they’d still leave behind rocks, gas and dust. With time that matter could come together again, making new stars and planets and maybe life.
Vacuum decay is different. This cataclysm would result from a change in the Higgs field, a quantum field that pervades all of space. It would be triggered by pure chance, creating a bubble that would expand at almost the speed of light, transforming all in its path. Inside that bubble the laws of physics we take for granted would change, making matter as we know it (and, consequently, life) impossible.
According to physicists’ current best estimates, vacuum decay is extremely unlikely, with an almost unthinkably small chance of its taking place close enough to our part of the universe to affect us. Still, the chance isn’t zero, and some recent estimates suggest the likelihood might be slightly less minuscule than we used to think. Ultimately, though, the possibility of an apocalyptic quantum bubble shouldn’t cause anyone to lose any sleep.
On supporting science journalism
If you’re enjoying this article, consider supporting our award-winning journalism by subscribing. By purchasing a subscription you are helping to ensure the future of impactful stories about the discoveries and ideas shaping our world today.
Even so, scientists have been studying how and why this scenario might play out. The answers to these questions don’t just reveal some fascinating aspects of the quantum world—they may also turn the questions on their heads: rather than making us worry about the threat a vacuum bubble poses, the fact that the universe has survived this long without one may teach us something about the deepest unsolved problems in physics.
The word “vacuum” evokes the idea of empty space, and that’s not too far from its meaning in the phrase “vacuum decay.” For physicists, however, “empty” itself is relative.
All the objects we’re used to—every animal, vegetable and mineral—are made up of atoms, and those atoms are made up of ripples in quantum fields. Each field is like a setting on a kind of universal control panel. If you could jiggle the electron switch on the control panel, you’d see an electron pop into existence. Most of these switches have a default value of zero: electrons aren’t likely to be in most places, for example. These defaults are sticky—it takes effort, in the form of energy, to push a switch out of its default position. How much energy it requires is determined by Albert Einstein’s famous equation E = mc2, which defines the relation between energy and mass: the more massive a particle, the stickier the default for the switch of its field.
Inside the bubble the laws of physics we take for granted would change.
You might think that in truly empty space, all these switches are set to zero. That’s true for most quantum fields, but some have a different default. One such case is a quantum field proposed by several physicists in 1964, including British physicist Peter Higgs, for whom it was later named. Try to set the Higgs field to zero, and it will resist. The universe “wants” to have a certain amount of Higgsness in it, a default called a vacuum expectation value. It is this amount of Higgs field, instead of zero, that one finds in the vacuum of empty space.
Pushing the Higgs field from this default setting is quite difficult. Scientists finally accomplished it in 2012, when an experiment at the Large Hadron Collider (LHC) near Geneva managed to measure the tiniest, briefest possible shift in the Higgs field. Just as jiggling the electron switch makes an electron, jiggling the Higgs switch makes a particle called a Higgs boson. These particles swiftly vanish after we create them, with the Higgs switch rushing back to its default while knocking other, easier-to-shift switches around, creating particles such as electrons or photons instead. But LHC scientists managed to create enough Higgs bosons to definitively detect them and prove the Higgs field exists.
The Higgs field is special because it controls the mass of all other particles. In effect, it serves as a kind of master switch, determining how sticky all the other switches’ defaults are. If you could grab the Higgs switch and drag it toward zero, you’d find that all the other switches became much easier to flick. In other words, a lower Higgs value would mean it took less energy to make an electron or a quark.
Physicists think of the task of moving the Higgs field from its default value as being a bit like rolling a boulder up a hill. If the boulder rests at the bottom of a valley, you can try to push it upward, but if you let it go, it will just roll down again.
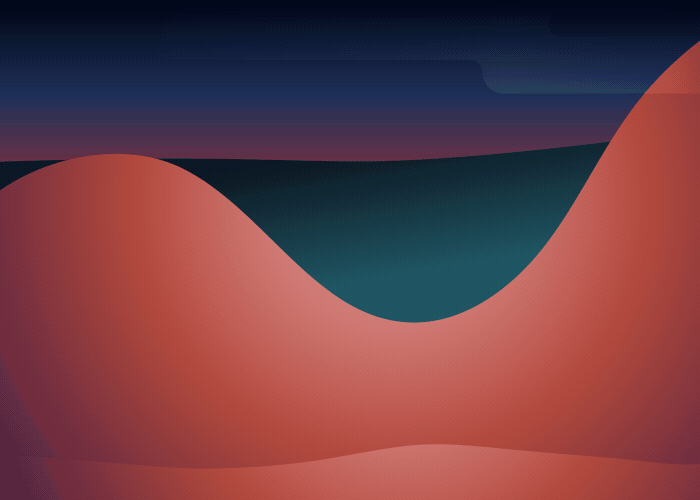
For the Higgs theory to work, the Higgs field needs to have a setting that is hard to change, near the bottom of its metaphorical valley. But the theory doesn’t say much about the world outside the valley.
Since the 1970s physicists have speculated that there could be a lower valley farther out corresponding to an even higher setting for the Higgs field. If it exists, that setting would be the true default, and ours would merely be a “false vacuum”—a temporary value that isn’t the one the Higgs field naturally wants to have. In the true vacuum, the Higgs field would be stronger, which would make other fundamental particles such as electrons much, much more massive and harder to create, upsetting the balance that lets atoms exist.
This other valley might seem almost impossible to get to. We would have to roll our metaphorical boulder up another hill before it passed the peak and started to roll down the other side to the lower valley. But quantum physics can make the impossible possible. Through an effect called quantum tunneling, a field can randomly jump from a higher-energy setting to a lower-energy one even if it lacks the energy to climb the hill in between. It’s as if it tunneled through the hill rather than traveling over it. If this happened to the Higgs field, it would end our false vacuum and make the true vacuum emerge.
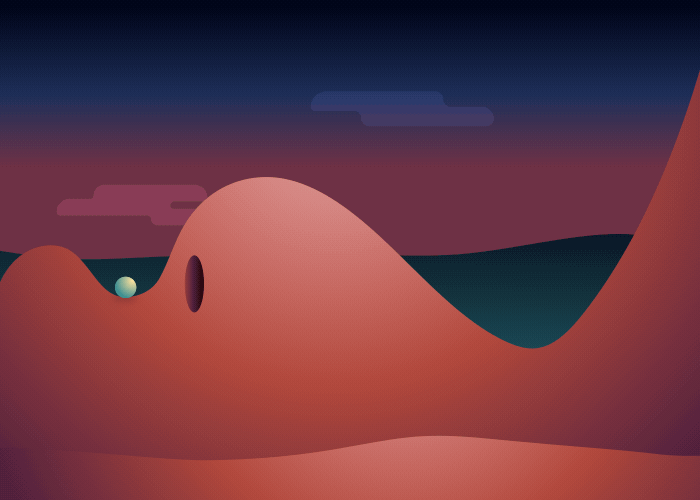
Physicists aren’t completely sure what would happen if this change, called vacuum decay, took place. Estimates suggest it would start in a small area, forming a bubble in which the Higgs field sat at its higher setting and all other particles had much higher masses. If the bubble were relatively small, it would wink out, pinched shut by a force akin to the surface tension of a drop of water. If the bubble were big enough, however, the huge difference in energy between the inside and outside would make it start to grow. In empty space, it would expand at the speed of light, changing the Higgs field’s setting across the cosmos. “It was all very theoretical until the Higgs boson was discovered,” says Matthew D. Schwartz, a professor of physics at Harvard University. Before then, no one knew the mass of the particle.
As a result, physicists weren’t sure vacuum decay was even possible. They had a formula to estimate its chance, but it depended on the difficulty of changing the Higgs from its current default setting, which was unknown. The formula said that if the switch were very sticky, our vacuum would be the correct default, not a false vacuum, and would never decay. If it were easy to shift, decay would be much more likely. When the LHC teams announced their discovery of the Higgs particle, we finally had a clear measurement of just how hard it is to shift the Higgs field. For the first time it was possible to calculate how likely vacuum decay should be.
The result was reassuring. In 2017 a team, including Schwartz, calculated a probability of just one in 10606 that a vacuum decay bubble would have reached us by now, an absurdly low chance. Imagine taking every atom from all the stars in the sky, giving each one its own universe of stars and then doing that again five more times. Now imagine someone selects one atom at random out of the final total, and you are asked to guess which it is; you would be more likely to pick that specific atom from those universes of universes than to experience vacuum decay.
There is, however, some uncertainty in the calculation, and physicists continue to update their estimates with new data. The formula used to compute the probability of vacuum decay depends not only on the difficulty of changing the Higgs field but also on the mass of other fundamental particles, as well as the strengths of the forces acting on those particles. A 2024 calculation based on more precise measurements lowered the chance even further to around one in 10868.
It’s possible that other factors affect the likelihood of vacuum decay. To understand why, think of throwing a pinch of salt into a pot of water just before it boils. When the salt hits the water, the few tentative bubbles that might have been brewing multiply into a froth of activity. If the water wasn’t boiling before, it is now. Physicists say the salt “nucleates” bubbles. Each grain of salt can serve as a seed, a little nucleus of change. The rough surface of the salt grains makes it easier for bubbles to form. Soon those bubbles spread until the whole pot is boiling.
As it turns out, what works for bubbles of steam also works for bubbles of vacuum. But you need fancier salt. Specifically, you need black holes.
In 2015 three physicists in England—Ruth Gregory and Philipp Burda, both at Durham University, and Ian Moss of Newcastle University—worked out what was needed. (Others had previously speculated that black holes might trigger vacuum decay, but with data from the LHC it was finally possible to run the numbers.) The U.K. team found that black holes can make vacuum decay dramatically more likely by seeding bubbles in the same way salt does in boiling water. To have an impact, though, the black holes need to be extremely tiny.
Most of the black holes that astronomers see in the universe are dead stars. When the biggest stars reach the end of their lives, they explode in supernovae that create black holes. Left alone, such a black hole will start to shrink. Stephen Hawking figured out that black holes diminish over time, releasing particles called Hawking radiation. This radiation happens because the extreme curvature of space and time around a black hole changes how quantum fields wiggle, turning a momentary jiggling of a switch into a long-lasting particle. Black hole evaporation is extremely slow for big, gently curved black holes, taking much longer than the age of the universe. As a black hole gets smaller, its curves become sharper, causing it to produce more and more particles and to evaporate faster and faster. The smallest black holes evaporate in just a blink of an eye.
We do have one key piece of evidence that tells us quite a bit: we’re alive.
Gregory and her collaborators found that the curvier spacetime was around a black hole, the bigger an effect it had on vacuum decay, for much the same reason: strongly curved spacetime makes it easier for quantum fields to change, including the Higgs field. Black holes made from stars are too big, and spacetime around them is too gently curved, to make vacuum decay noticeably more likely. Black holes that are very small won’t matter, either, because they evaporate before they have a chance to cause a problem. But black holes that are somewhere in between, with masses of ounces or so, can stir up bubbles.
Making a black hole that small would require compressing an ounce of material into a space many times smaller than a proton, something that can’t happen now either technologically or astrophysically. But close to the time of the big bang, black holes might have formed before stars existed as extremely hot, dense matter rippled and cooled. These primordial black holes could have been the right size to seed vacuum decay bubbles, or they could have shrunk to the right size later. Astronomers have searched for evidence of small primordial black holes because they could also explain the mysterious phenomenon of dark matter. So far no evidence for them has been found.
In 2019 Gregory teamed up with two U.S.-based physicists, De-Chang Dai of Case Western Reserve University and Dejan Stojkovic of the State University of New York at Buffalo. Together they figured out how many tiny black holes would be needed to destroy the universe. The topic fascinated Stojkovic. “I hate to speculate without calculations, but once you have calculations, as crazy as it looks, you have to face it,” he says. “You have to start taking it seriously. What if there is a bubble near Earth? And the bubble is moving with the speed of light, so we’d better calculate this very quickly!”
Once when Stojkovic presented the team’s vacuum decay findings at a meeting in Florida, a curmudgeon in the audience asked why he should care. If vacuum bubbles expand at the speed of light, one would hit us before we noticed it was coming. In that case, what use is there in knowing about it?
The question motivated Stojkovic to look deeper. Working with Dai again, as well as Djordje Minic of Virginia Tech, he found that although a vacuum bubble will travel at the speed of light in empty space, it gets slowed down when it encounters massive objects such as stars and planets. This year Stojkovic, Dai and a student of Stojkovic’s named Amartya Sengupta published a preprint paper entitled “The Signals of the Doomsday,” in which they describe what astronomers might see if such a bubble were nearby: a blast of light with a particular spectrum. What if we see such a signal? “Then you get to decide what to do,” Stojkovic says. “I don’t know—just go to the beach?”
A more imaginative option was explored by Ashoke Sen, a physicist at the International Center for Theoretical Sciences in Bangalore. In 2015 Sen published an essay proposing that humanity could survive vacuum decay by riding the expansion of space itself. Spacetime is always expanding, and that expansion appears to be speeding up because of a mysterious phenomenon called dark energy. As space pulls apart faster and faster, distant places will be carried apart faster than the speed of light. Sen suggested that if humanity could spread far enough among the stars early enough in cosmic time, it would guarantee that at least some people would escape death by vacuum decay. The expanding universe would drive them apart faster than any onrushing bubble of Higgs-based doom. The paper appeared online on April Fool’s Day, but Sen pursued the idea further later that year, which suggests he takes it at least somewhat seriously.
There is a lot physicists still don’t know about vacuum decay. Better measurements from particle colliders could change calculations dramatically, as could finding evidence for tiny black holes. But there is an even bigger mystery, one we probably won’t be able to solve anytime soon.
When physicists calculate the chance of vacuum decay, they’re using a theory called the Standard Model of particle physics. The Standard Model includes every known particle and describes the results of experiments with remarkable precision. But we know it’s incomplete. The model breaks when physicists try to use it to describe particles with an extremely high energy called the Planck energy. If we could collide two protons with this energy, which is roughly equal to the energy in a full tank of gas for a typical car, we couldn’t predict what would happen: the Standard Model would give us nonsense.
Something new has to be added to the Standard Model to fix it. Physicists expect there to be new fields: new settings for the universe that we haven’t noticed because they take vast amounts of energy to change. These fields would correspond to extremely massive particles that we haven’t discovered yet. If these new fields affect the Higgs, though, then all bets are off. The existence of such new fields and particles might change the odds of vacuum decay occurring, and they could even mean there is no second valley for the Higgs after all.
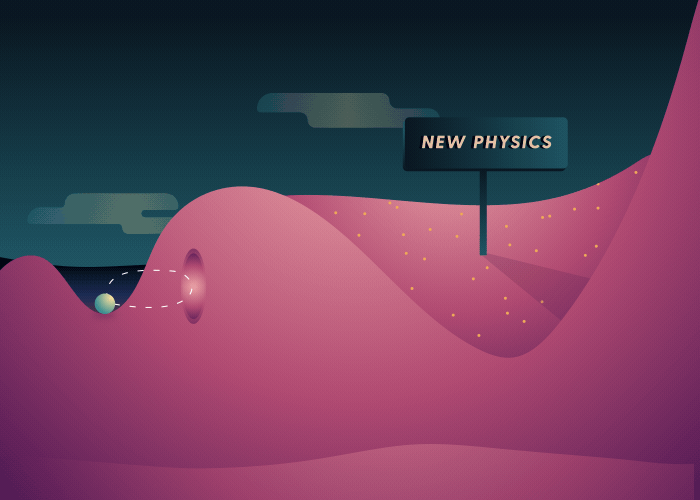
At this point you might think we know practically nothing about vacuum decay. But we do have one key piece of evidence that tells us quite a bit: we’re alive. The simple fact that vacuum decay hasn’t occurred already—that the universe has been allowed to carry on for 13.7 billion years without an annihilating bubble sweeping through it—puts limits on how likely vacuum decay can be.
Suppose physicists calculated, based on the Standard Model, that there is a 90 percent chance that a vacuum bubble would have found us by now. That might sound scary, but think a minute. Essentially those same odds would have applied to the past as well. A thousand years ago, for instance, we would already have had an 89.999999 percent chance of being hit by a vacuum bubble (the odds then were slightly lower because the universe was slightly younger, meaning a bubble would have had slightly less time to reach us). Clearly, we haven’t been wiped out, so that 90 percent calculation can’t be the entire story. “The universe would be unstable, and that would mean there would have to be new physics to stabilize it. Otherwise we wouldn’t be here,” Schwartz says.
Our very existence can teach us something about the laws of physics far beyond what we can test. If physicists calculated a high chance of vacuum decay, and yet vacuum decay still hadn’t happened, it would all but guarantee that the Higgs field’s faraway second valley has a bundle of new quantum fields in it that the Standard Model doesn’t take into account. We can’t measure those fields now, and we won’t be able to soon. But in a utopian future among the stars, maybe we will. And calculations we do now could tell our descendants where to look.